Thank you for visiting nature.com. You are using a browser version with limited support for CSS. To obtain the best experience, we recommend you use a more up to date browser (or turn off compatibility mode in Internet Explorer). In the meantime, to ensure continued support, we are displaying the site without styles and JavaScript.
Scientific Reports volume 6, Article number: 20024 (2016 ) Cite this article Protease Inhibitor
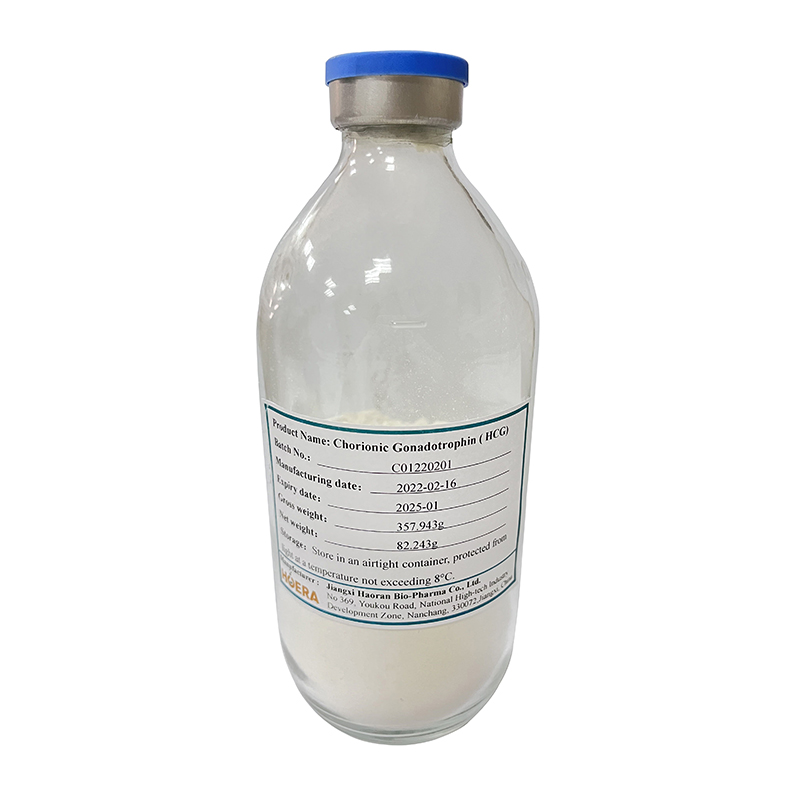
An Erratum to this article was published on 29 April 2016
This article has been updated
The tissue kallikrein-related peptidase family (KLK) is a group of trypsin- and chymotrypsin-like serine proteases that share a similar homology to parent tissue kallikrein (KLK1). KLK1 is identified in heart and has anti-hypertrophic effects. However, whether other KLK family members play a role in regulating cardiac function remains unknown. In the present study, we demonstrated for the first time that KLK8 was expressed in myocardium. KLK8 expression was upregulated in left ventricle of cardiac hypertrophy models. Both intra-cardiac adenovirus-mediated and transgenic-mediated KLK8 overexpression led to cardiac hypertrophy in vivo. In primary neonatal rat cardiomyocytes, KLK8 knockdown inhibited phenylephrine (PE)-induced cardiomyocyte hypertrophy, whereas KLK8 overexpression promoted cardiomyocyte hypertrophy via a serine protease activity-dependent but kinin receptor-independent pathway. KLK8 overexpression increased epidermal growth factor (EGF) production, which was blocked by the inhibitors of serine protease. EGF receptor (EGFR) antagonist and EGFR knockdown reversed the hypertrophy induced by KLK8 overexpression. KLK8-induced cardiomyocyte hypertrophy was also significantly decreased by blocking the protease-activated receptor 1 (PAR1) or PAR2 pathway. Our data suggest that KLK8 may promote cardiomyocyte hypertrophy through EGF signaling- and PARs-dependent but a kinin receptor-independent pathway. It is implied that different KLK family members can subtly regulate cardiac function and remodeling.
Wenyu Fu, Dmytro Vasylyev, ... Chuan-ju Liu
Xuhui Bao, Yongjun Liang, … Liyi Xie
Nicola Alesi, Damir Khabibullin, … Elizabeth P. Henske
The tissue kallikrein-related peptidase family (KLK) is a group of trypsin- and chymotrypsin-like serine proteases that share a similar homology to parent tissue kallikrein (KLK1)1. KLKs are encoded by tandemly arranged genes of multigene families, which comprise 15 genes in human and 20 genes in rat2. KLKs are translated as pre-proenzymes and then cleaved upon release from the secretary pathway to become proenzymes. Once secreted, pro-KLKs are further processed by other KLKs or other proteases to become active extracellular enzymes1,2. Kininogen is a well-known substrate of KLK members. Low-molecular weight kininogen is cleaved by KLK1, leading to the release of the vasoactive peptide kinin1,2. Kinin interacts with the kinin receptors to exert a broad spectrum of biological effects such as, vasodilation3, non-vascular smooth muscle contraction4,5 and inflammation6,7. Besides kininogens, a variety of substrates can be degraded by KLK family serine protease activity. For instance, extracellular matrix proteins including collagen and fibronectin can be degraded by both KLK family members1,2,8,9. The protelytic process of pro-epidermal growth factor (EGF) into mature EGF is mediated by KLK110. In addition, protease-activated receptors (PARs) are also indentified to be the substrates of KLK family members10,11,12.
KLK8, which is also known as neuropsin, is a new member of KLKs. It was first identified in the mouse hippocampus13. KLK8 has now been identified in a variety of tissues, with highest density in skin and brain14. As a member of KLKs, KLK8 may exert its function by cleaving various proteins as other KLK members. Although the substrates for KLK8 remain largely unknown, it has been shown that the effect of KLK8 on skin desquamation15, synaptic plasticity in brain16 and invasiveness of tumor cells17 is associated with its serine protease activity.
Among KLKs, KLK1 is the most widely studied member. In myocardium, a local KLK1-kinin system has been identified18,19. Kinins have protective effects against myocardial ischemia/reperfusion insult or hypertrophy by interacting with two specific receptors, kinin B1 receptor (B1R) and B2 receptor (B2R)20,21,22. Abnormality in KLK1–kinin system (KKS) is associated with cardiac hypertrophy2,23. However, whether KLK8 is expressed in myocardium and its functions in the heart have not been reported. Here, we identified the expression of KLK8 in rat myocardium and investigated the expression pattern of KLK8 in hypertrophic hearts. We further explored the effects of KLK8 on cardiomyocyte hypertrophy and underlying mechanisms both in vitro and in vivo.
As shown in Fig. 1A, immunoreactive KLK8 was localized in ventricular myocytes of rat heart using a primary antibody that recognizes KLK8. KLK8 Immunoreactivity was abolished when the antibody was preabsorbed with excess peptide, thereby confirming the specificity of the antibody.
KLK8 is upregulated in hypertrophic hearts.
(A) Expression of KLK8 in myocardium. The left panel showed negative control. Pre-absorption of the KLK8 primary antibody was performed with a 10-fold excess of the blocking peptide. The right panel is the representative immunostained section of rat left ventricular myocardium with antibody against KLK8. The positive staining was mainly localized to cardiomyocytes as indicated by arrows. Original magnification, ×400. Scale bars correspond to 100 μm. (B,C) showed levels of cardiac hypertrophic markers (HW/BW, ANP, BNP and Myh7) and KLK8 in myocardium of Sham and TAC rats, respectively. D and E showed levels of cardiac hypertrophic markers (ANP, BNP and Myh7) and KLK8 in myocardium of WKY and SHR rats, respectively. F and G showed KLK1 levels in myocardium of Sham/TAC and WKY/SHR rats, respectively. Data were expressed as mean percentage of Sham or WKY ± SEM (n = 7). *P < 0.05, **P < 0.01 vs Sham. #P < 0.05, ##P < 0.01 vs WKY. Representative Western blotting bands are on the top of corresponding graphs.
We then investigated the expression pattern of KLK8 in hypertrophic hearts. Compared to the sham-operated rats, 8-week TAC rats showed significant left ventricular hypertrophy as evidenced by 1.5-fold increased heart weight to body weight (HW/BW) ratio, 2.3-fold increased ANP mRNA expression, 2.4-fold increased ANP mRNA expression and 1.9-fold increased Myh7 mRNA expression (Fig. 1B). The mRNA and protein levels of KLK8 were significantly increased in the hearts subjected to TAC compared with Sham group (Fig. 1C, P < 0.05).
It is known that hypertension could result in left ventricular hypertrophy. In spontaneously hypertensive rats (SHR), hypertrophy changes were confirmed by 3.7-fold increased ANP, 2.7-fold increased of BNP and 2.1-fold increased Myh7 mRNA expression as compared with Wister Kyoto (WKY) rats (Fig. 1D). Myocardium KLK8 expression was also higher in SHR rats compared with WKY rats (Fig. 1E, P < 0.01).
Given that KLK1 is also involved in cardiac hypertrophy, the expression of KLK1 in hypertrophic hearts was also examined. As shown in Fig. 1F,G, KLK1 mRNA and protein levels were decreased in myocardium of TAC rats compared with controls. However, there is no significant change in KLK1 mRNA and protein levels in SHR hearts compared with WKY hearts.
As KLK8 expression was increased in hypertrophy heart tissues, we then investigated whether in vivo intra-cardiac adenovirus-mediated KLK8 delivery might lead to cardiac hypertrophy. Two weeks after injection of Ad-KLK8 into the anterior wall of left ventricle (LV), KLK8 expression was confirmed by western blotting. As shown in Fig. 2A, KLK8 expression was robustly increased in the anterior wall of LV tissue and KLK8 expression was not changed in the posterior wall of LV and the right ventricle. Four weeks after intra-cardiac injection of Ad-vector and Ad-KLK8, the anterior wall of LV tissue was obtained for measurement of cardiac hypertrophy markers and histological analysis. It was found that intra-cardiac KLK8 gene delivery led to a significantly increase in the transcripts of cardiac hypertrophy markers including ANP and Myh7 (Fig. 2B). Analysis of WGA-stained heart sections revealed that the anterior wall of LV tissue obtained from rats injected with Ad-KLK8 have approximately 1.3-fold increase in cross-sectional area of cardiomyocytes as compared with those obtained from rats injected with Ad-control (Fig. 2C,D). Masson’s staining showed that the extent of cardiac fibrosis was comparable in LV tissues obtained from rats injected with Ad-KLK8 or control adenovirus (Fig. 2E).
Intra-cardiac injection of Ad-KLK8 leads to cardiac hypertrophy.
(A) Representative western blot analysis of KLK8 protein expression in the anterior wall of left ventricle (LV), posterior wall of LV and the right ventricle two weeks after intra-cardiac injection of Ad-KLK8 and Ad-control into the anterior wall of LV. B-F, Four weeks after intra-cardiac injection of Ad-KLK8 and Ad-control, experimental animals were used for measurements of cardiac hypertrophic markers (B), cross-sectional area (C,D), interstitial fibrosis (E), as well as echocardiography analysis (F). (B) mRNA level of cardiac hypertrophic markers (ANP and Myh7) in the anterior wall of LV was determined by quantitative real-time RT-PCR. (C) WGA staining was performed on transverse sections of the anterior wall of LV. (D) Mean cardiomyocyte cross-sectional area was quantified using the Image-J cell area measurement software. Six rats were analyzed for each group and 30 to 40 cardiomyocytes were measured per rat (n = 200 cells/group). (E) Histological analysis using masson staining. F, Representative M-mode images of rats subjected to intra-cardiac injection of Ad-control or Ad-KLK8. Scale bar: 50 μm. ** vs Ad-vector.
Echocardiographic analysis was performed to independently measure the diameter of the LV and the thickness of the ventricular walls, as well as LV function 4 weeks after intra-cardiac injection with Ad-KLK8 or control adenovirus. Figure 2F showed representative M-mode echocardiographic images of the hearts. We found that the diameter of the LV was decreased significantly in rats injected with Ad-KLK8 compared with those injected with control adenovirus (Table 1). Notably, the thickness of the anterior LV wall was significantly increased in rats injected with Ad-KLK8 compared with those injected with control adenovirus. The thickness of posterior LV wall was not differed between Ad-KLK8 and control adenovirus mice. LV functions characterized by LV ejection fraction and LV fractional shortening were significantly enhanced in rats injected with Ad-KLK8 compared with those injected with control adenovirus.
To further define the role of KLK8 overexpression in vivo, we established KLK8 transgenic rats. In four independent lines, all of the rats were born normally and survived to adulthood and were fertile. KLK8 is overexpressed in the heart in all lines of Tg-KLK8 rats (Fig. 3A). The relative levels of KLK8 expression in the different lines were line No. 7 > 24 > 29 > 13. In this study, we presented the results of experiments in Tg-KLK8 line 7, which showed the highest expression level of KLK8 in the heart. As shown in Fig. 3 and Table 2, the KLK8 transgenic rats developed significant cardiac hypertrophy at both 6-weeks and 12-weeks of age. They showed higher left ventricle weight/body weight (LVW/BW) and heart weight/body weight (HW/BW) ratios than the littermate controls (Table 2). The transcripts of cardiac hypertrophy markers including ANP, BNP and Myh7 (Fig. 3B–D) were also significantly up-regulated in the heart tissues of KLK8 transgenic rats. Quantitative measurement of 6-weeks-old and 12-weeks-old KLK8 transgenic cardiomyocytes using WGA staining revealed 1.37-fold and 1.33-fold increase in cell cross-sectional area as compared to 6-weeks-old and 12-weeks-old control rats, respectively (Fig. 3E,F). Masson’s staining showed that the extent of cardiac fibrosis was comparable in LV tissues obtained from 6-weeks-old KLK8 transgenic rats or 6-weeks-old control rats (Fig. 3G). However, the area of fibrosis seen on Masson’s trichrome staining was significantly increased in LV tissues obtained from 12-weeks-old KLK8 transgenic rats compared with those obtained from 12-weeks-old control rats (Fig. 3G).
KLK8 transgenic rats show cardiac dysfunction.
(A) Western blot analysis showed significant increases of KLK8 protein expression in heart homogenates obtained from Tg-KLK8 rats compared with those from control rats. (B–D) mRNA level of cardiac hypertrophic markers ANP (B), BNP (C) and Myh7 (D) in hearts from Tg-KLK8 and littermate control rats at the age of 6 and 12 weeks were determined by quantitative real-time RT-PCR. (E) WGA staining was performed on transverse sections of the hearts from Tg-KLK8 and littermate control rats at the age of 6 and 12 weeks. (F) Mean cardiomyocyte cross-sectional area was quantified using the Image-J cell area measurement software. Six rats were analyzed for each group and 30 to 40 cardiomyocytes were measured per rat (n = 200 cells/group). (G) Histological analysis using masson staining of the hearts from Tg-KLK8 and littermate control rats at the age of 6 and 12 weeks. H, Representative M-mode images of Tg-KLK8 and littermate control rats at the age of 6 and 12 weeks. Scale bar: 50 μm. *P < 0.05, **P < 0.01 vs 6-week old littermate control rats; #P < 0.05, ##P < 0.01 vs 12-week old littermate control rats.
To evaluate the effect of transgenic overexpression of KLK8 on cardiac function, we first assessed the LV dimensions and functions of 6-weeks-old and 12-weeks-old KLK8 transgenic rats by using M-mode echocardiography. As shown in Table 2, thickening of the LV walls, as well as a decrease in the LV internal diameter in diastole, were shown in KLK8 transgenic rats as compared with the littermate control rats, at both 6-weeks and 12-weeks of age. Notably, both ejection fraction and fractional shortening were increased in 6-weeks-old KLK8 transgenic rats, however were decreased in 12-weeks-old KLK8 transgenic rats, as compared with the littermate control rats.
Functional invasive hemodynamic measurements were performed to confirm the occurrence of echocardiographic alterations in KLK8 transgenic rats (Table 2). There was no significant difference in heart rates between the control and transgenic rats. Peak rates of ventricular contraction (dP/dtmax) and relaxation (dP/dtmin) were increased in 6-weeks-old KLK8 transgenic rats, however were decreased in 12-weeks-old KLK8 transgenic rats, as compared with the littermate control rats. These results reflected the progressive cardiac dysfunction in KLK8 transgenic rats.
To further confirm the role of KLK8 in cardiomyocyte hypertrophy, KLK8 was overexpressed in cultured cardiomyocytes with KLK8 adenovirus. As shown in Fig. 4A, infection of cardiomyocytes with KLK8 adenovirus resulted in KLK8 overexpression. Overexpression of KLK8 per se significantly increased cardiomyocyte protein content (Fig. 4B), cell size (Fig. 4C) and transcripts of ANP, BNP and Myh7 (Fig. 4D–F) in cardiomyocytes. We also found that KLK8 expression in cardiomyocytes was significantly induced by PE treatment. Moreover, Ad-KLK8 and PE treatment had an additive effect on KLK8 expression (Fig. 4A). As shown in Fig. 4B–F, KLK8 overexpression substantially aggravated PE-induced cardiomyocyte hypertrophy.
KLK8 induces cardiomyocyte hypertrophy and aggravates PE-induced cardiomyocyte hypertrophy in vitro.
Primary cultured neonatal cardiomyocytes were infected with KLK8 adenovirus. After incubation for 72 h, cells were treated with PE for another 48 h. (A) showed KLK8 expression level in cardiomyocytes treated by KLK8 adenovirus with or without PE. (B) total protein content was determined by BCA assay; (C) Cardiomyocytes were stained with FITC-conjugated phalloidin as described in “Methods”. One hundred cells from randomly selected fields in three independent expreriments were evaluated for cell size using the Image-Pro Plus cell area measurement software. Representative images are on the top of the corresponding graph. (D–F) transcripts of cardiac hypertrophy markers including ANP (D), BNP (E) and Myh7 (F) were determined by quantitative real-time RT-PCR. Values are presented as mean ± SEM (n = 3). Scale bar: 50 μm. **P < 0.01 vs cells treated with Ad-vector; ##P < 0.01 vs cells treated with Ad-vector and PE.
As KLK8 expression is increased in hypertrophy heart tissues, we then investigated whether endogenous KLK8 is involved in cardiomyocyte hypertrophy. As shown in Fig. 5A, transfection of siRNA targeting KLK8 not only resulted in approximately 70% decrease in basal KLK8 protein expression, but also significantly decreased PE-induced KLK8 expression in cardiomyocytes. PE caused an increase in protein content, cell size and the transcripts of hypertrophy markers including ANP, BNP and Myh7 in cultured cardiomyocytes (Fig. 5B–F). KLK8 siRNA partly reversed the PE-induced hypertrophy as showing decreased protein content, cell size and the mRNA levels of ANP, BNP and Myh7. KLK8 siRNA didn’t affect the basal levels of above parameters in cultured cardiomyocytes.
Knockdown of KLK8 inhibits PE-induced cardiomyocyte hypertrophy.
Primary cultured neonatal cardiomyocytes were transfected with control siRNA or KLK8 siRNA for 24 h and then treated with PE for another 48 h. (A) showed KLK8 protein expression. (B) total protein content was determined by BCA assay; (C), Cardiomyocytes were stained with FITC-conjugated phalloidin as described in “Methods”. One hundred cells from randomly selected fields in three independent expreriments were evaluated for cell size using the Image-Pro Plus cell area measurement software. (D–F) transcripts of cardiac hypertrophy markers including ANP (D), BNP (E) and Myh7 (F) were determined by quantitative real-time RT-PCR. Values are presented as mean ± SEM (n = 3). *P < 0.05, **P < 0.01 vs cells treated with Control siRNA; #P < 0.05, ##P < 0.01 vs cells treated with Control siRNA and PE.
We then investigated the molecular mechanisms responsible for KLK8 induction of hypertrophy. KLK family is supposed to cleave kininogens to release kinin. Kinin is well known to have protective effect in cardiomyocytes by interacting with kinin receptors1,2. We found that infection of cardiomyocytes with KLK8 adenovirus resulted in a 3.56 ± 0.58 fold increase in the content of bradykinin in culture media. However, neither kinin B1R antagonist R715 nor kinin B2R antagonist HOE140 reversed the hypertrophic effects of KLK8 (Fig. 6B–F). To further confirm the role of kinin receptors in KLK8 induced cardiomyocytic hypertrophy, we used siRNA approach to knockdown kinin B1R and B2R (Supplementary Fig. 1A,B). It was found that knockdown of B1R or B2R could not reverse KLK8-induced hypertrophy (Fig. 6B–F). As shown in Supplementary Fig. 2 and 3, neither antagonists nor knockdown of kinin B1/B2 receptors reversed the additive effect of Ad-KLK8 and PE on cardiomyocytic hypertrophy.
KLK8-induced cardiomyocyte hypertrophy is not dependent on kinin B1R and B2R.
Primary cultured neonatal cardiomyocytes were infected with KLK8 adenovirus, 24 h later control siRNA, kinin B1R siRNA or B2R siRNA was transfected into the cardiomyocytes. Twenty four hours later, all the media of cells were replaced with fresh media, then kinin B1R antagonist R715, or kinin B2R antagonist HOE140 was added into the culture media, After incubation for 24 h, cells were treated with PE for another 48 h. (A) showed KLK8 protein expression; (B) total protein content was determined by BCA assay; (C) Cardiomyocytes were stained with FITC-conjugated phalloidin as described in “Methods”. One hundred cells from randomly selected fields in three independent expreriments were evaluated for cell size using the Image-Pro Plus cell area measurement software. (D–F) transcripts of cardiac hypertrophy markers including ANP (D), BNP (E) and Myh7 (F) were determined by quantitative real-time RT-PCR. Values are presented as mean ± SEM (n = 3). **P < 0.01 vs cells treated with Ad-vector and control siRNA.
As KLK8 is a member of trypsin- and chymotrypsin-like serine proteases, we then investigated whether the hypertrophic effect of KLK8 is through its proteolytic activity. Two serine protease inhibitors, antipain and ZnSO4 were used to block the proteolytic activity of KLK8 as described previously8. As shown in Fig. 7, antipain and ZnSO4 entirely blocked the hypertrophic effects of KLK8. In addition, the additive effect of Ad-KLK8 on PE-induced cardiomyocytic hypertrophy was abolished by antipain and ZnSO4 (Supplementary Fig. 4). Taken together, these findings suggest that the hypertrophic effect of KLK8 is dependent on its proteolytic activty
Serine protease inhibitor entirely blocks the hypertrophic effects of KLK8.
Primary cultured neonatal cardiomyocytes were infected with KLK8 adenovirus, 24 h later serine protease inhibitor antipain or ZnSO4 was added into the culture media. After incubation for 24 h, cells were treated with PE for another 48 h. (A) showed KLK8 protein expression; (B) total protein content was determined by BCA assay; (C) Cardiomyocytes were stained with FITC-conjugated phalloidin as described in “Methods”. One hundred cells from randomly selected fields in three independent expreriments were evaluated for cell size using the Image-Pro Plus cell area measurement software. (D–F) transcripts of cardiac hypertrophy markers including ANP (D), BNP (E) and Myh7 (F) were determined by quantitative real-time RT-PCR. Values are presented as mean ± SEM (n = 3). **P < 0.01 vs cells treated with Ad-vector; ##P < 0.01vs cells treated with Ad-KLK8.
KLKs have the ability to hydrolyze a variety of substrates such as pro-epidermal growth factor (pro-EGF). It is well known that EGF induces hypertrophy in various cell types24,25. Thus, we investigated whether the hypertrophic effect of KLK8 was through releasing EGF. As shown in Fig. 8A, it was found that overexpression of KLK8 in cardiomycytes significantly increased the EGF level in the culture media. This effect was completely abolished by serine protease inhibitors antipain and ZnSO4. Neither pharmacological antagonists nor siRNAs of kinin B1 and B2 receptors affected EGF level in KLK8 overexpressed cells.
The hypertrophic effect of KLK8 is dependent on EGF signaling.
(A) Primary cultured neonatal cardiomyocytes were infected with KLK8 adenovirus, 24 h later control siRNA, kinin B1R siRNA or B2R siRNA was transfected into the cardiomyocytes. Twenty four hours later, all the media of cells were replaced with fresh media, then serine protease inhibitors (antipain or ZnSO4), kinin B1R antagonist R715, or kinin B2R antagonist HOE140 was added into the culture media, After incubation for 24 h, culture media were collected for determination of EGF. (B–F) Cells were infected with KLK8 adenovirus, 24 h later control siRNA or EGFR siRNA was transfected into the cardiomyocytes. Twenty four hours later, all the media of cells were replaced with fresh media, then EGFR antagonist AG1478 was added into the culture media. The hypertrophic effects were detected after additional 48 h of incubation. B showed KLK8 protein expression. Total protein content was determined by BCA assay (C). (D) Cardiomyocytes were stained with FITC-conjugated phalloidin as described in “Methods”. One hundred cells from randomly selected fields in three independent expreriments were evaluated for cell size using the Image-Pro Plus cell area measurement software. Transcripts of cardiac hypertrophy markers including ANP (E), BNP (F) and Myh7 (G) were determined by quantitative real-time RT-PCR. Values are presented as mean ± SEM (n = 3). **P < 0.01 vs cells treated with Ad-vector and control siRNA; ##P < 0.01 vs cells treated with Ad-KLK8 and control siRNA.
To confirm EGF is involved in hypertrophic effect of KLK8, the effects of the antagonist of EGF receptor and siRNA targeting EGF receptor (EGFR) on KLK8-induced cardiomyocyte hypertrophy were then investigated. As shown in Fig. 8C–G, EGF receptor antagonist blocked hypertrophic effects of KLK8. In addition, we also used EGFR siRNA, which resulted in 70% reduction of EGFR (Supplementary Fig. 1C). It was found that knockdown of EGFR also significantly attenuated KLK8-induced hypertrophy.
We next examined whether EGF signaling contributed to the hypertrophic effect of KLK8 in vivo. As shown in Fig. 9, it was found that administration of EGFR antagonist (3 mg/kg) significantly attenuated the hypertrophic effects of intra-cardiac Ad-KLK8 gene delivery, as evidenced by the decreases in the transcripts of cardiac hypertrophy markers (Fig. 9A–C) and cross-sectional area of cardiomyocytes (Fig. 9D,E) as compared with those obtained from rats injected with Ad-KLK8 alone.
Administration of EGFR antagonist significantly attenuated the hypertrophic effects of intra-cardiac Ad-KLK8 gene delivery in vivo.
Adenovirus particles containing KLK8 or control vector were administered to rats by intra-cardiac injection into the anterior wall of left ventricular. Rats injected by Ad-KLK8 were then treated by selective EGFR antagonist AG1478 (3 mg/kg/day, i.p.). Two weeks after intra-cardiac injection of Ad-KLK8 and Ad-control, experimental animals were used for measurements of cardiac hypertrophic markers (A–C) and cross-sectional area (D,E). (A–C) mRNA level of cardiac hypertrophic markers ANP (A), BNP (B) and Myh7(C) in the anterior wall of LV was determined by quantitative real-time RT-PCR. (D) WGA staining was performed on transverse sections of the anterior wall of LV. (E) Mean cardiomyocyte cross-sectional area was quantified using the Image-J cell area measurement software. Six rats were analyzed for each group and 30 to 40 cardiomyocytes were measured per rat (n = 200 cells/group). Scale bar: 50 μm. *P < 0.05, **P < 0.01 vs Ad-vector; #P < 0.05, ##P < 0.01 vs Ad-KLK8.
KLKs have been demonstrated to regulate cell signaling by cleaving and activating the protease-activated receptors (PARs), which have been implicated in many cellular effects including hypertrophy development26,27. Thus, we investigated whether PARs contribute to the hypertrophic effect of KLK8. As shown in Fig. 10, both PAR1 antagonists RWJ56110 and PAR2 antagonist FSLLRY-NH2 significantly attenuated the hypertrophic effects of KLK8. In addition, we also used siRNAs targeting PAR1 and PAR2, which resulted in 80% and 85% reduction of PAR1 and PAR2, respectively (Supplementary Fig. 1D,E). It was found that knockdown of either PAR1 or PAR2 also blocked KLK8-induced hypertrophy (Fig. 10).
Both PAR1 and PAR2 are involved in the hypertrophic effect of KLK8.
Primary cultured neonatal cardiomyocytes were infected with KLK8 adenovirus, 24 h later control siRNA, PAR1 siRNA or PAR2 siRNA was transfected into the cardiomyocytes. Twenty four hours later, all the media of cells were replaced with fresh media, then PAR1 antagonist RWJ56110, or PAR2 antagonist FSLLRY-NH2 was added into the culture media. After incubation for 24 h, cells were treated with PE for another 48 h. (A) showed KLK8 protein expression. (B) total protein content was determined by BCA assay; (C) Cardiomyocytes were stained with FITC-conjugated phalloidin as described in “Methods”. One hundred cells from randomly selected fields in three independent expreriments were evaluated for cell size using the Image-Pro Plus cell area measurement software. (D–F) transcripts of cardiac hypertrophy markers including ANP (D), BNP (E) and Myh7 (F) were determined by quantitative real-time RT-PCR. Values are presented as mean ± SEM (n = 3). *P < 0.05, **P < 0.01 vs cells treated with Ad-vector and control siRNA; #P < 0.05, ##P < 0.01vs cells treated with Ad-KLK8 and control siRNA.
In the in vivo studies, it was found that administration of PAR1 antagonists RWJ56110 (1 mg/kg) and PAR2 antagonist FSLLRY-NH2 (1 mg/kg) significantly attenuated the hypertrophic effects of intra-cardiac Ad-KLK8 gene delivery, as evidenced by the decreases in the transcripts of cardiac hypertrophy markers (Fig. 11A–C) and cross-sectional area of cardiomyocytes (Fig. 11D,E) as compared with those obtained from rats injected with Ad-KLK8 alone.
Administration of PAR1 or PAR2 antagonist significantly attenuated the hypertrophic effects of intra-cardiac Ad-KLK8 gene delivery in vivo.
Adenovirus particles containing KLK8 or control vector were administered to rats by intra-cardiac injection into the anterior wall of left ventricular. Rats injected by Ad-KLK8 were then treated by selective PAR1 antagonist RWJ56110 (1 mg/kg/day, i.p.) or PAR2 antagonist FSLLRY-NH2 (1 mg/kg/day, i.p.). Two weeks after intra-cardiac injection of Ad-KLK8 and Ad-control, experimental animals were used for measurements of cardiac hypertrophic markers (A–C) and cross-sectional area (D,E). (A–C) mRNA level of cardiac hypertrophic markers ANP (A), BNP (B) and Myh7(C) in the anterior wall of LV was determined by quantitative real-time RT-PCR. (D) WGA staining was performed on transverse sections of the anterior wall of LV. (E) Mean cardiomyocyte cross-sectional area was quantified using the Image-J cell area measurement software. Six rats were analyzed for each group and 30 to 40 cardiomyocytes were measured per rat (n = 200 cells/group). Scale bar: 50 μm. *P < 0.05, **P < 0.01 vs Ad-vector; #P < 0.05, ##P < 0.01 vs Ad-KLK8.
Previous studies have demonstrated that EGFR and PARs activate some common effector pathways such as PLC, ERK1/2, p38 MAPK and Akt28,29. Among these signaling molecules, ERK1/2 is known to be involved in cardiac hypertrophy-induced by both EGFR and PARs. Mechanically, the ERK1/2 pathway induces cardiac hypertrophy may, at least in part, by enhancing the transcriptional activity of NFκB, an important hypertrophic transcription factor30. Thus, we next investigated whether KLK8 activated ERK1/2 and NFκB via EGFR and PAR in vivo. As shown in Fig. 12, we found that intra-cardiac injection of KLK8 resulted in significant increases of phosphor-ERK1/2 and phosphor-NFκB p65 subunit levels, which were attenuated by administration of EGFR antagonist, PAR1 antagonist, or PAR2 antagonist.
Intra-cardiac injection of KLK8 resulted in significant increases of phosphor-ERK1/2 and phosphor-NFκB levels, which were attenuated by administration of EGFR antagonist, PAR1 antagonist, or PAR2 antagonist.
Adenovirus particles containing KLK8 or control vector were administered to rats by intra-cardiac injection into the anterior wall of left ventricular. Rats injected by Ad-KLK8 were then treated by selective EGFR antagonist AG1478 (3 mg/kg/day, i.p.), PAR1 antagonist RWJ56110 (1 mg/kg/day, i.p.), or PAR2 antagonist FSLLRY-NH2 (1 mg/kg/day, i.p.). Two weeks after intra-cardiac injection of Ad-KLK8 and Ad-control, levels of phosphor-ERK1/2 and phosphor-NFκB p65 subunit in the anterior wall of LV was determined by Western blot analysis. *P < 0.05, **P < 0.01 vs Ad-vector; ##P < 0.01 vs Ad-KLK8.
The present study demonstrates for the first time that KLK8 is expressed in cardiomyocytes. Furthermore, we provide the evidence that KLK8 induces hypertrophy in cardiomyocytes both in vivo and in vitro.
Previous studies have demonstrated that KLK1 reduces cardiac hypertrophy and fibrosis in various cardiac hypertrophy models31,32,33,34,35. KLK1 acts mainly on low-molecular weight kininogen to produce kinin peptides, which exert anti-hypertrophic effects mainly via kinin B2R31,34. Kinins are continuously released during cardiac hypoxia, ischemia and myocardial infarction36. Cardiac KLK1-kinin system is implicated to be a prime mediator in protecting the heart in hypertrophic conditions37. It is known that kinin peptides consist of bradykinin and kallidin in humans or bradykinin and the kallidin-like peptide in rodents38. All of these peptides could interact with kinin B1R and B2R. In addition, it has been shown that, in kninogen deficient rats, KLK1 can reduce cardiomyocytic apoptosis and improve cardiac performance of infarcted hearts via kinin B2R, suggesting that KLK1 may release “kinin-like peptides” not from kininogen39. In the present study, we found that KLK8 could release bradykinin in cardiomyocytes, meanwhile significantly promote cardiac hypertrophy. These differences in KLK1 and KLK8 effects suggest that KLK1 and KLK8 may release different peptides, which have different effects on hypertrophy.
Besides kininogens, a variety of substrates can be degraded by KLK family serine proteases. For instance, both KLK1 and KLK8 degrade extracellular matrix proteins including collagen and fibronectin1,2,8,9. Guillon-Munos A et al.40 have indicated that KLK12 induces hydrolysis of matricellular proteins of the CCN (cyr61, ctgf, nov) family which contributes to the bioavailability and/or activity of several growth factors including VEGF, BMP2, TGF-β1 and FGF-2. More recently, Sanchez WY et al.41 showed that selective cleavage of human sex hormone-binding globulin (SHBG) by active KLK4 and KLK14 affects androgen action in LNCaP prostate cancer cells. In the present study, we found that the hypertrophic effect of KLK8 was entirely abolished by inhibition of KLK8 serine protease activity, but was not affected by either pharmacological blockade or siRNA-mediated knock-down of kinin B1R and B2R. These results indicate that KLK8 induction of hypertrophy be not through releasing kinin peptides but is dependent on its serine protease activity. Notably, a limitation of this study is that KLK8 proteolytic activity was not assessed in KLK8 overexpression cells since it is difficult to evaluate KLK8 activity on the cellular level. To date, gelatin zymography is the most usually used method to determine proteolytic activity of KLK family members including KLK842. As gelatin is the common substrate for KLK family members43, this method is not specific for KLK8 and mainly used to assess recombinant KLK8 activity in vitro. However, as mentioned, we showed that the inhibitors of serine protease reversed hypertrophy induced by KLK8 overexpression.
EGF plays an important role in promoting hypertrophic responses in cardiac pathologies24,25. It has been shown that active EGF signaling pathway can be induced by KLK family members in a variety of cell types. For example, KLK1 cleaved pro-epidermal growth factor (EGF) into mature EGF, which finally activated EGF receptor (EGFR) signaling pathway and led to hypertrophy of primary cultured tracheal submucosal gland cells10. More recently, Abdallah et al.44 demonstrated in primary vascular smooth muscle cells that plasma kallikrein was capable of stimulating a disintegrin and metalloprotease (ADAM) 17 activity and leading sequentially to the release of the endogenous EGF receptor ligand amphiregulin which contributed to the transactivation of EGF receptor and ERK1/2 activation. We found that overexpression of KLK8 led to an increase in EGF, which could be abolished by serine protease inhibitors. In addition, blockade of EGF receptor reversed KLK8-induced hypertrophy both in vitro and in vivo. These results suggest that KLK8 promote cardiomyocyte hypertrophy via activation of EGF signaling pathway.
PARs are well-known to be activated by thrombin or other coagulation or inflammatory proteases formed at sites of tissue injury45. PAR1 activation triggers a range of signaling events in cardiomyocytes that lead to changes in electrical/mechanical function45. Cardiomyocyte-specific overexpression of PAR1 in mice induces eccentric hypertrophy and dilated cardiomyopathy27. As for PAR-2, it activates a spectrum of biochemical and functional responses that largely mimic cardiomyocyte activation by PAR-1 (including PLC, ERK, p38-MAPK, increased [Ca2+]i, enhanced spontaneous automaticity and elongated/dilated hypertrophy) in cardiomyocytes46. KLKs are recently known to regulate cell function by cleaving and activating members of PAR family. For example, KLK4 and KLK14 mediate PAR1 and PAR2 activation in colon cancer cells, respectively47,48. KLK6 is an activator of PAR1/2 in neurons and astrocytes49. Whether KLK8 activates PAR1/2 signaling remains unknown. The present study found that both PAR1/2 inhibitor and PAR1/2 knockdown significantly reversed KLK8-induced hypertrophy in cardiomyocytes. In addition, administration of PAR1 or PAR2 inhibitor significantly attenuated the hypertrophic effects of intra-cardiac Ad-KLK8 gene delivery. These data provided convincing evidence that PAR1/2 activation contributed to KLK8-induced cardiac hypertrophy. However, the limitation was that we didn’t perform biochemical experiments to investigate how KLK8 cleaved and activated PAR1 and PAR2 in cardiomyocytes. The precise mechanisms involved in PAR1/2 activation by KLK8 should be considered in future studies.
EGFR and PARs are known to activate some common effector pathways such as PLC, ERK1/2, p38 MAPK and Akt28,29. Among these signaling molecules, ERK1/2 is known to be involved in cardiac hypertrophy-induced by both EGFR and PARs. Mechanically, the ERK1/2 pathway induces cardiac hypertrophy may, at least in part, by enhancing the transcriptional activity of NFκB30. The present study found that intra-cardiac injection of KLK8 resulted in significant increases of phosphor-ERK1/2 and phosphor-NFκB levels, which were attenuated by administration of EGFR antagonist, PAR1 antagonist, or PAR2 antagonist. Taken together, these results suggest that ERK1/2-NFκB signaling pathway may be the downstream effector of EGFR and PAR1/2 and play a critical role in the hypertrophic effect of KLK8.
Cardiac ryanodine receptor (RyR2) macromolecular complex, a large ion channel composed of four 560-kDa RyR subunits and four 12.6-kDa FK506 binding proteins (FKBP12.6, also known as calstabin2), is a major determinant of intracellular Ca2+ release in cardiomyocytes and required for excitation-contraction coupling50. Growing evidence indicates that disturbances of Ca2+ dynamics due to dysfunction of RyR2 macromolecular complex results in several cardiac disorders including cardiac hypertrophy and heart failure50. For example, recent studies show that genetic deletion or dysfunction of calstabin2 can lead to cardiac hypertrophy and dysfunction51. Furthermore, previous reports indicated that calstabin1 (also known as FKBP12), which shares 85% sequence identity with calstabin2, can form an endogenous inhibitor of EGFR phosphorylation directly involved in the control of cellular EGFR activity52. Thus, it is of interest to investigate whether calstabin2 contributes to KLK8-induced cardiac hypertrophy and dysfunction through modulating EGFR activity.
It is well-recognized that intrinsic cardiac aging leads to structural and functional deteriorations of the heart in elderly individuals. Cardiac hypertrophy is a hallmark of cardiac aging53. Accumulating evidence shows that aging results in an increase in the prevalence of left ventricular hypertrophy, a decline in diastolic function and relatively preserved systolic function at rest but a decline in exercise capacity51,53. Recent studies have shown the involvements of multiple molecular mechanisms in the development of cardiac aging. In particular, an imbalance of synthesis and degradation of extracellular matrix proteins has been implicated in the pathogenesis of aging-associated cardiac hypertrophy54. Since KLK family members have been observed to be involved in cardiac hypertrophy through modulating ECM synthesis and degradation, whether KLK8 plays a role in aging-associated cardiac hypertrophy merits further investigation.
As a selective α1-adrenoceptor agonist, phenylephrine has been reported to mediate cardiac hypertrophy and aortic contractile responses55. The present study found that PE could promote expression of KLK8 in neonatal myocytes. KLK8 knockdown partly reversed the PE-induced hypertrophy, whereas PE and KLK8 overexpression have an additive effect on cardiomyocyte hypertrophy. In the presence of two protease inhibitors, antipain and ZnSO4, cardiomyocytes treated with PE plus KLK8 overexpression showed no significant differences on hypertrophic markers as compared with cells treated with PE only, indicating that protease inhibitors abolished the additive effect of KLK8 overexpression on PE-induced hypertrophy. Taken together, these results let us suggest that the involvement of KLK8 in PE-induced cardiomyocytic hypertrophy might dependent on the serine proteolytic activity of KLK8.
As mentioned before, KLK proteins are secreted as enzymatically inactive proKLKs, which are further processed by other KLKs or other proteases to become active enzymes1,2. It has been demonstrated that KLK family members can co-localize in same tissue and biological fluid and can be activated by each other through an orchestrated proteolytic cascades56,57. For example, KLK5 autoactivates and then can activate the pro-forms of KLK1, KLK6, KLK8 and KLK11 etc. More recently, it has been reported that the active KLK8 could target pro-KLK1 and pro-KLK1142. In the present study, it is uncertain whether KLK8 exerts its functions through activating other KLKs, such as KLK1, or KLK1 exerts its function through KLK8. However, our data indicate that at least two KLK family member are co-expressed in cardiomyocytes and have effects on hypertrophy.
We found that KLK1 and KLK8 showed different expression pattern in hypertrophic myocardium. In TAC-induced hypertrophy, KLK8 was up-regulated whilst KLK1 was down-regulated. Given that KLK8 induces hypertrophy whereas KLK1 inhibits hypertrophy, upregulation of KLK8 and downregulation of KLK1 would exacerbate the progress of hypertrophy. Our data that there was no significant difference in KLK1 expression in myocardium between SHR and WKY rats are not consistent with other studies where it has been demonstrated that cardiac KLK1 activity is significantly decreased in SHR rats as compared to WKY rats58. However, their study did not show the protein level of KLK1. It is known that serine protease activity does not always parallel its protein level. Nevertheless, the differences in KLK1 and KLK8 expression pattern in cardiac hypertrophy suggest that KLK1 and KLK8 might play different roles in this cardiac disorder. The molecular mechanisms responsible for modulating KLK1 and KLK8 levels in the pathogenesis of hypertrophy remain to be investigated.
The present study demonstrates that KLK8, a new member of KLK family, is expressed in cardiomycytes and can induce cardiac hypertrophy. KLK8 promotes hypertrophy through EGF and PAR1/2 signaling-dependent and kinin receptor-independent signaling mechanisms. It is implied that different KLK members subtle regulate cardiac function and remodeling.
Male Sprague-Dawley rats (8-week-old), SHR rats (16-week-old) and WKY rats (16-week-old) were obtained from Shanghai SLAC Laboratory Animal Co (Shanghai, China) and housed at controlled room temperature with free access to food and water under a natural day/night cycle. All animal protocols were approved by the Ethical Committee of Experimental Animals of Second Military Medical University and confirmed to the principal in the Guide for the Care and Use of Laboratory Animals published by the United States National Institute of Health (NIH publication No. 85-23, revised 1996).
Left ventricular hypertrophy was induced by abdominal TAC in male SD rats as described previously34. Briefly, the aorta was exposed through a midline abdominal incision. A blunt 21-gauge needle was then placed adjacent to the abdominal aorta above the renal arteries. A ligature was tightened around the aorta and the needle was removed after ligation. Sham-operated rats underwent the same surgical procedure without the actual ligation. Rats were sacrificed at 56 days after aortic constriction.
Paraffin heart sections (5 μm) were stained with KLK8 (sc-67666, Santa Cruz Biotechnology) or Masson trichrome (to examine interstitial fibrosis) as previously described59. To confirm the specificity of primary antibody of KLK8, pre-absorption of the primary antibody with a 10-fold excess of the blocking peptides sc-67666 P (Santa Cruz Biotechnology, Inc.) was performed as negative controls. To measure myocyte cross-sectional area, we used fluorescence-tagged wheat germ agglutinin (WGA) staining (5.0 μg/mL; with samples incubated in the dark for 10 minutes at 37 °C). Images were recorded at 494-nm excitation and 518-nm emission and were evaluated with ImageJ60.
KLK8 adenovirus was generated by using the AdEasyTM adenoviral vector system (Stratagene, La Jolla, CA, USA). Rat KLK8 recombinant adenoviruses were finally harvested, purified and tittered by standard methods61. An empty adenoviral construct used at the same titer served as control. The details are described in the Supplementary materials and methods. The overexpression of KLK8 was achieved by infecting cultured neonatal rat ventricular cardiomyocytes with the recombinant adenovirus (multiplicity of infection [MOI] = 10). For in vivo gene delivery, 5 × 1011 adenovirus particles containing KLK8 or control vector were administered by direct intra-cardiac injection into the anterior wall of left ventricular (5 sites, 50 μl/site), using a syringe with a 30-gauge needle62,63.
F0 transgenic Sprague-Dawley rat embryos were generated by pronuclear injection of the expression vector containing the rat KLK8 cDNA by Cyagen Biosciences Inc. (Guangzhou, China). Founders and the offspring were maintained on a 12 h light/dark cycle and fed standard rat chow ad libitum in the Animal Research Center of Second Military Medical University. Genotyping of KLK8 transgenic rats was determined by PCR screening of genomic DNA extracted from rat-tail biopsies using two primer pairs called CMV-F/KLK8-R and KLK8-F/IRES-R. Primer sequences were shown in Supplementary Table 2.
Hemodynamic parameters and cardiac function were measured in 6-week and 12-week ages of Tg-KLK8 rats and the wild type littermates. Briefly, rats underwent left ventricular catheterization via left common carotid artery. Hemodynamic variables were measured with a pressure transducer and recorded on a BL-420 S Biologic Function Experiment system (Taimeng technology, Chengdu, China).
Echocardiography was performed using a 7.5-MHz phased-array transducer (Acuson Sequoia 256, Siemens, Mountain View, CA) at 28 days after intra-myocardial KLK8 gene delivery as previously described64. Left-ventricular end-diastolic and -systolic internal diameter (LVIDd and LVIDs, respectively), left-ventricular end-diastolic and -systolic anterior wall thickness (LVAWd and LVAWs, respectively) and left-ventricular end-diastolic and -systolic posterior wall thickness (LVPWd and LVPWs, respectively) were measured. The cardiac systolic function was indicated by fractional shortening (FS) and ejection fraction (EF). All measurements represented the mean values of the signals from three consecutive cardiac cycles and were carried out by two experienced technicians who were unaware of the identities of the respective experimental groups.
Ventricle myocytes were isolated from rats that were up to 3 days old and were isolated and cultured as described previously65. Briefly, ventricle tissues were minced and serially digested. Cell pellets were resuspended and placed in culture dishes at 37 °C for 1 hour to allow selective attachment of nonmyocytes. Cardiomyocyte-enriched fraction were then seeded at a density of 1 × 105 cells/cm2 and cultured in DMEM containing 15 mmol/L HEPES, 10% FBS, 0.1 mmol/L bromodeoxyuridine (BrdU) and antibiotics (100 U/mL penicillin and 100 mg/mL streptomycin) for 48 hours. The culture medium was then exchanged for serum-free DMEM containing the same additives with the exception of BrdU. The details are described in the Supplementary materials and methods.
The small interfering RNA (siRNA) for KLK8, B1R, B2R, ERGR, PAR1, PAR2 were designed and synthesized by GenePharma Corporation (Shanghai, China). Transfection of siRNA was performed by using siPORT NeoFx transfection agent (Ambion , Austin, TX) according to the instructions of the manufacturer. The details are described in the Supplementary materials and methods.
Total RNA from heart tissue or cardiomyocytes was extracted and then reverse transcribed to generate cDNA. Quantitative real-time PCR was carried out using SYBRGreen (F Hoffmann-La Roche Ltd, Basel, Switzerland) as detection dye. The housekeeping gene glyceraldehyde-3-phosphate dehydrogenase (GAPDH) was measured for each sample as an internal control for sample loading and normalization. The sequences of PCR products ascertain that their identities matched to the known sequences of rat KLK8, ANP, BNP and Myh7. The details are described in the Supplementary materials and methods.
Proteins of rat heart tissues and cardiomyocytes were extracted using cold T-Per and M-Per lysis buffer (Pierce Biotechnology), respectively. Protein samples were separated by SDS-PAGE and subsequently transferred to nitrocellulose membranes. All antibodies were purchased from Santa Cruz Biotechnology (Santa Cruz Biotechnology, Inc. Santa Cruz, CA). The details are described in the Supplementary materials and methods.
For cell surface area measurement, cardiomyocytes were plated at a density of 5 × 104 cells/3 cm dish to obtain individually plated cells. At the end of the treatment period, the cardiomyocytes were fixed in PBS plus 4% formaldehyde. After permeabilization with 0.1% Triton X-100 in PBS, fixed cells were incubated 3 h at room temperature with FITC-conjugated phalloidin (1:100, sigma) in PBS containing 1% BSA to visualize myocyte sarcomeres (F-actin). Images were acquired using an inverted Olympus fluorescence microscope. Cell images from six randomly chosen fields (40×objective), representing four separate experiments, were measured using the Image-Pro Plus cell area measurement software and the percentage of mean cell surface area normalized to control group were calculated. Only cells lying completely within the field were quantified.
The amounts of bradykinin and EGF in the incubation medium of primary cultured cardiomyocytes were assayed using the commercially available EIA kit (Phoenix Pharmaceuticals, Belmont, CA) and ELISA kit (Peprotech, Rocky Hill, NJ), respectively.
Adenovirus particles containing KLK8 or control vector were administered to rats by intra-cardiac injection into the anterior wall of left ventricular. Rats injected by Ad-KLK8 were then treated by selective EGFR antagonist AG1478 (3 mg/kg/day, i.p.), PAR1 antagonist RWJ56110 (1 mg/kg/day, i.p.), or PAR2 antagonist FSLLRY-NH2 (1 mg/kg/day, i.p.) for 2 weeks.
All data were expressed as means ± SEM. For illustrative purposes, some results are presented as the mean percent control ± SEM. When comparing multiple groups, one-way or two-way ANOVA was performed and when significant (p < 0.05), comparisons between each groups were conducted using the Student-Newman-Keuls test. SPSS 13.0 statistical software was used for data analysis. P < 0.05 was considered statistically significant.
How to cite this article: Cao, B. et al. Kallikrein-related peptidase 8 is expressed in myocardium and induces cardiac hypertrophy. Sci. Rep. 6, 20024; doi: 10.1038/srep20024 (2016).
A correction has been published and is appended to both the HTML and PDF versions of this paper. The error has been fixed in the paper.
Lundwall, A. & Brattsand, M. Kallikrein-related peptidases. Cell Mol Life Sci. 65, 2019–2038 (2008).
Chao, J. et al. Tissue kallikrein in cardiovascular, cerebrovascular and renal diseases and skin wound healing. Biol Chem. 391, 345–355 (2010).
Bergaya, S., Matrougui, K., Meneton, P., Henrion, D. & Boulanger, C. M. Role of tissue kallikrein in response to flow in mouse resistance arteries. J Hypertens. 22, 745–750 (2004).
Akbar, A., Sharma, J. N., Yusof, A. P. & Gan, E. K. Potentiation of bradykinin-induced responses by indomethacin in the intact and denuded epithelium of guinea pig tracheal preparations. Int J Tissue React. 20, 95–100 (1998).
Andrade, S. S. et al. A plant Kunitz-type inhibitor mimics bradykinin-induced cytosolic calcium increase and intestinal smooth muscle contraction. Biol Chem. 393, 943–957 (2012).
Stadnicki, A. Intestinal tissue kallikrein-kinin system in inflammatory bowel disease. Inflamm Bowel Dis. 17, 645–654 (2011).
Viel, T. A. & Buck, H. S. Kallikrein-kinin system mediated inflammation in Alzheimer’s disease in vivo. Curr Alzheimer Res. 8, 59–66 (2011).
Kishi, T., Cloutier, S. M., Kündig, C., Deperthes, D. & Diamandis, E. P. Activation and enzymatic characterization of recombinant human kallikrein 8. Biol Chem. 387, 723–731 (2006).
Rajapakse, S., Ogiwara, K., Takano, N., Moriyama, A. & Takahashi, T. Biochemical characterization of human kallikrein 8 and its possible involvement in the degradation of extracellular matrix proteins. FEBS Lett. 579, 6879–6884 (2005).
Casalino-Matsuda , SM , Monzon , ME , Conner , GE , Salathe , M. & Forteza , RM Role of hyaluronan and reactive oxygen species in tissue kallikrein-mediated epidermal growth factor receptor activation in human airways .J Biol Chem 279, 21606–21616.
Vandell, A. G. et al. Protease-activated receptor dependent and independent signaling by kallikreins 1 and 6 in CNS neuron and astroglial cell lines. J Neurochem. 107, 855–870 (2008).
CAS PubMed PubMed Central Google Scholar
Ramsay, A. J. et al. Hooper JD. Kallikrein-related peptidase 4 (KLK4) initiates intracellular signaling via protease-activated receptors (PARs). KLK4 and PAR-2 are co-expressed during prostate cancer progression. J Biol Chem. 283, 12293–12304 (2008).
Chen, Z. L. et al. Expression and activity-dependent changes of a novel limbic-serine protease gene in the hippocampus. J Neurosci. 15, 5088–5097 (1995).
Yoshida, S. Klk8, a multifunctional protease in the brain and skin: analysis of knockout mice. Biol Chem. 391, 375–380 (2010).
Kishibe, M. et al. Kallikrein 8 is involved in skin desquamation in cooperation with other kallikreins. J Biol Chem. 282, 5834–5841 (2007).
Shiosaka, S. & Ishikawa, Y. Neuropsin–a possible modulator of synaptic plasticity. J Chem Neuroanat. 42, 24–29 (2011).
Sher, Y. P. et al. Human kallikrein 8 protease confers a favorable clinical outcome in non-small cell lung cancer by suppressing tumor cell invasiveness. Cancer Res. 66, 11763–11770 (2006).
Emanueli, C. & Madeddu, P. Role of the kallikrein-kinin system in the maturation of cardiovascular phenotype. Am J Hypertens. 12, 988–999 (1999).
Trabold, F. et al. Cardiovascular phenotypes of kinin B2 receptor- and tissue kallikrein-deficient mice. Hypertension. 40, 90–95 (2002).
Yoshida, H., Zhang, J. J., Chao, L. & Chao, J. Kallikrein gene delivery attenuates myocardial infarction and apoptosis after myocardial ischemia and reperfusion. Hypertension. 35, 25–31 (2000).
Yin, H., Chao, L. & Chao, J. Kallikrein/kinin protects against myocardial apoptosis after ischemia/reperfusion via Akt-glycogen synthase kinase-3 and Akt-Bad.14-3-3 signaling pathways. J Biol Chem. 280, 8022–8030 (2005).
Yin, H., Chao, L. & Chao, J. Nitric oxide mediates cardiac protection of tissue kallikrein by reducing inflammation and ventricular remodeling after myocardial ischemia/reperfusion. Life Sci 82, 156–165 (2008).
Moreau, ME et al.The kallikrein-kinin system: current and future pharmacological targets.J Pharmacol Sci.99, 6–38 (2005).
Purdom, S. & Chen, Q. M. Epidermal growth factor receptor-dependent and –independent pathways in hydrogen peroxide-induced mitogen-activated protein kinase activation in cardiomyocytes and heart fibroblasts. J Pharmacol Exp Ther 312, 1179–1186 (2005).
Rebsamen, M. C., Arrighi, J. F., Juge-Aubry, C. E., Vallotton, M. B. & Lang, U. Epidermal growth factor induces hypertrophic responses and Stat5 activation in rat ventricular cardiomyocytes. J Mol Cell Cardiol. 32, 599–610 (2000).
Antoniak, S., Sparkenbaugh, E. M., Tencati, M., Rojas, M., Mackman, N. & Pawlinski, R. Protease activated receptor-2 contributes to heart failure. PLoS One. 8, e81733 (2013).
ADS PubMed PubMed Central Google Scholar
Pawlinski, R. et al. Protease-activated receptor-1 contributes to cardiac remodeling and hypertrophy. Circulation. 116, 2298–2306 (2007).
CAS PubMed PubMed Central Google Scholar
Steinberg, S. F. The cardiovascular actions of protease-activated receptors. Mol Pharmacol. 67, 2–11 (2005).
Shah, B. H. & Catt, K. J. Matrix metalloproteinase-dependent EGF receptor activation in hypertension and left ventricular hypertrophy. Trends Endocrinol Metab. 15, 241–243 (2004).
Huang, H., Joseph, L. C., Gurin, M. I., Thorp, E. B. & Morrow, J. P. Extracellular signal-regulated kinase activation during cardiac hypertrophy reduces sarcoplasmic/endoplasmic reticulum calcium ATPase 2 (SERCA2) transcription. J Mol Cell Cardiol. 75, 58–63 (2014).
CAS PubMed PubMed Central Google Scholar
Batista, E. C. et al. Swimming training exacerbates pathological cardiac hypertrophy in kinin B2 receptor-deficient mice. Int Immunopharmacol 8, 271–275 (2008).
Bledsoe, G., Chao, L. & Chao, J. Kallikrein gene delivery attenuates cardiac remodeling and promotes neovascularization in spontaneously hypertensive rats. Am J Physiol Heart Circ Physiol 285, H1479–H1488 (2003).
Chao, J., Zhang, J. J., Lin, K. F. & Chao, L. Human kallikrein gene delivery attenuates hypertension, cardiac hypertrophy and renal injury in Dahl salt-sensitive rats. Hum Gene Ther. 9, 21–31 (1998).
Li, H. J. et al. Tissue kallikrein protects against pressure overload-induced cardiac hypertrophy through kinin B2 receptor and glycogen synthase kinase-3beta activation. Cardiovasc Res. 73, 130–142 (2007).
Silva, J. A. Jr. et al. Reduced cardiac hypertrophy and altered blood pressure control in transgenic rats with the human tissue kallikrein gene. FASEB J 14, 1858–1860 (2000).
Pan, H. L., Chen, S. R., Scicli, G. M. & Carretero, O. A. Cardiac interstitial bradykinin release during ischemia is enhanced by ischemic preconditioning. Am J Physiol Heart Circ Physiol 279, H116–H121 (2000).
Sharma, J. N. Role of tissue kallikrein-kininogen-kinin pathways in the cardiovascular system. Arch Med Res. 37, 299–306 (2006).
ADS CAS PubMed Google Scholar
Kakoki, M. & Smithies, O. The kallikrein-kinin system in health and in diseases of the kidney. Kidney Int 75, 1019–1030 (2009).
CAS PubMed PubMed Central Google Scholar
Chao, J. et al. Tissue kallikrein elicits cardioprotection by direct kinin b2 receptor activation independent of kinin formation. Hypertension. 52, 715–720 (2008).
CAS PubMed PubMed Central Google Scholar
Guillon-Munos, A. Kallikrein-related peptidase 12 hydrolyzes matricellular proteins of the CCN family and modifies interactions of CCN1 and CCN5 with growth factors. J Biol Chem. 286, 25505–25518 (2011).
CAS PubMed PubMed Central Google Scholar
Sanchez, W. Y. Selective cleavage of human sex hormone-binding globulin by kallikrein-related peptidases and effects on androgen action in LNCaP prostate cancer cells. Endocrinology. 153, 3179–3189 (2012).
Eissa, A., Amodeo, V., Smith, C. R. & Diamandis, E. P. Kallikrein-related peptidase-8 (KLK8) is an active serine protease in human epidermis and sweat and is involved in a skin barrier proteolytic cascade. J Biol Chem. 286, 687–706 (2011).
Pampalakis, G. & Sotiropoulou, G. Tissue kallikrein proteolytic cascade pathways in normal physiology and cancer. Biochim Biophys Acta. 1776, 22–31 (2007).
Abdallah, R. T. et al. Plasma kallikrein promotes epidermal growth factor receptor transactivation and signaling in vascular smooth muscle through direct activation of protease-activated receptors. J Biol Chem. 285, 35206–35215 (2010).
CAS PubMed PubMed Central Google Scholar
Steinberg, S. F. The cardiovascular actions of protease-activated receptors. Mol Pharmacol. 67, 2–11 (2005).
Sabri, A. et al. Signaling properties and functions of two distinct cardiomyocyte protease-activated receptors. Circ Res. 86, 1054–1061 (2000).
Gratio, V. et al. Kallikrein-related peptidase 4: a new activator of the aberrantly expressed protease-activated receptor 1 in colon cancer cells. Am J Pathol. 176, 1452–1461 (2010).
CAS PubMed PubMed Central Google Scholar
Gratio, V. et al. Kallikrein-related peptidase 14 acts on proteinase-activated receptor 2 to induce signaling pathway in colon cancer cells. Am J Pathol. 179, 2625–2636 (2011).
CAS PubMed PubMed Central Google Scholar
Vandell, A. G. et al. Protease-activated receptor dependent and independent signaling by kallikreins 1 and 6 in CNS neuron and astroglial cell lines. J Neurochem. 107, 855–870 (2008).
CAS PubMed PubMed Central Google Scholar
Phrommintikul, A. & Chattipakorn, N. Roles of cardiac ryanodine receptor in heart failure and sudden cardiac death. Int J Cardiol. 112, 142–152 (2006).
Yuan, Q. et al. Functional role of Calstabin2 in age-related cardiac alterations. Sci Rep. 4, 7425 (2014).
CAS PubMed PubMed Central Google Scholar
Mathea, S., Li, S., Schierhorn, A., Jahris, G. & Schiene-Fischer, C. Suppression of EGFR autophosphorylation by FKBP12.Biochemistry.50, 10844–10850 (2011).
Chiao, Y. A. & Rabinovitch, P. S. The Aging Heart. Cold Spring Harb Perspect Med. 5, a025148 (2015).
PubMed PubMed Central Google Scholar
Kwak, H. B. Aging, exercise and extracellular matrix in the heart. J Exerc Rehabil. 9, 338–347 (2013).
PubMed PubMed Central Google Scholar
García-Sáinz, J. A., Vázquez-Prado, J. & Villalobos-Molina, R. Alpha 1-adrenoceptors: subtypes, signaling and roles in health and disease. Arch Med Res. 30, 449–458 (1999).
Borgoño, C. A. et al. A potential role for multiple tissue kallikrein serine proteases in epidermal desquamation. J Biol Chem 282, 3640–3652 (2007).
Emami, N. & Diamandis, E. P. Human kallikrein-related peptidase 14 (KLK14) is a new activator component of the KLK proteolytic cascade. Possible function in seminal plasma and skin. J Biol Chem. 283, 3031–3041 (2008).
Sharma, J. N., Uma, K. & Yusof, A. P. Left ventricular hypertrophy and its relation to the cardiac kinin-forming system in hypertensive and diabetic rats. Int J Cardiol. 63, 229–235 (1998).
Zhang, Y. Q. et al. Resveratrol ameliorates lipopolysaccharide-induced epithelial mesenchymal transition and pulmonary fibrosis through suppression of oxidative stress and transforming growth factor-β1 signaling. Clin Nutr. 34, 752–760 (2015).
Santulli, G. et al. CaMK4 Gene Deletion Induces Hypertension. J Am Heart Assoc. 1, e001081 (2012).
PubMed PubMed Central Google Scholar
Nicklin S. A. & Baker A. H. Simple methods for preparing recombinant adenoviruses for high-efficiency transduction of vascular cells. Methods Mol Med. 30, 271–283 (1999).
Rengo, G. et al. Myocardial adeno-associated virus serotype 6-betaARKct gene therapy improves cardiac function and normalizes the neurohormonal axis in chronic heart failure. Circulation. 119, 89–98 (2009).
Chen, C. et al. Cytosolic CARP promotes angiotensin II- or pressure overload-induced cardiomyocyte hypertrophy through calcineurin accumulation. PLoS One. 9, e104040 (2014).
ADS PubMed PubMed Central Google Scholar
Ma, J. et al. Time course of myocardial stromal cell-derived factor 1 expression and beneficial effects of intravenously administered bone marrow stem cells in rats with experimental myocardial infarction. Basic Res Cardiol. 100, 217–223 (2005).
Jian X. et al. MiR-204 regulate cardiomyocyte autophagy induced by hypoxia-reoxygenation through LC3-II. Int J Cardiol. 148, 110–112 (2011).
The authors thank Jian-Guo Jia and Rui Chen (Shanghai Institute of Cardiovascular Diseases, Zhongshan Hospital of Fudan University) for their technical assistance of Echocardiography analysis. This work was supported by Major State Basic Research Program of China (No. 2013CB967404 to Xin Ni), National Natural Science Foundation of China (No. 31171120 & No. 31271270 to Xiao-Yan Zhu and No. 31571227 to Jianqiang Lu) and New Outstanding Young Scholar Program of Shanghai Health Bureau (XYQ2011045 to Xiao-Yan Zhu).
Cao Buqing, Yu Qing and Zhao Wei contributed equally to this work.
Department of Physiology, Second Military Medical University, Shanghai, 200433, China
Buqing Cao, Qing Yu, Wei Zhao, Binhai Cong, Jiankui Du, Xiaoyan Zhu & Xin Ni
School of Kinesiology, The key Laboratory of Exercise and Health Sciences of Ministry of Education, Shanghai University of Sport, Shanghai, 200438, China
Zhiping Tang & Jianqiang Lu
You can also search for this author in PubMed Google Scholar
You can also search for this author in PubMed Google Scholar
You can also search for this author in PubMed Google Scholar
You can also search for this author in PubMed Google Scholar
You can also search for this author in PubMed Google Scholar
You can also search for this author in PubMed Google Scholar
You can also search for this author in PubMed Google Scholar
You can also search for this author in PubMed Google Scholar
You can also search for this author in PubMed Google Scholar
X.N. and X.Z. conceived and designed the study. B.C. (Buqing Cao), Q.Y. and W.Z. performed all the experiments. Q.Y., Z.T., B.C. (Binhai Cong), J.L. and J.D. prepared the figures. X.N. and X.Z. wrote the manuscript. All authors have reviewed the manuscript.
The authors declare no competing financial interests.
This work is licensed under a Creative Commons Attribution 4.0 International License. The images or other third party material in this article are included in the article’s Creative Commons license, unless indicated otherwise in the credit line; if the material is not included under the Creative Commons license, users will need to obtain permission from the license holder to reproduce the material. To view a copy of this license, visit http://creativecommons.org/licenses/by/4.0/
Cao, B., Yu, Q., Zhao, W. et al. Kallikrein-related peptidase 8 is expressed in myocardium and induces cardiac hypertrophy. Sci Rep 6, 20024 (2016). https://doi.org/10.1038/srep20024
DOI: https://doi.org/10.1038/srep20024
Anyone you share the following link with will be able to read this content:
Sorry, a shareable link is not currently available for this article.
Provided by the Springer Nature SharedIt content-sharing initiative
By submitting a comment you agree to abide by our Terms and Community Guidelines. If you find something abusive or that does not comply with our terms or guidelines please flag it as inappropriate.
Scientific Reports (Sci Rep) ISSN 2045-2322 (online)

Luteal Phase Defect Sign up for the Nature Briefing newsletter — what matters in science, free to your inbox daily.